Timisoara_Med 2023, 2023(1), 5; doi:10.35995/20230105
Article
Influence of the Printing Angle on the Accuracy of 3D-Printed Interim Fixed Partial Dentures: An In Vitro Study
Department of Prosthodontics, Faculty of Dental Medicine, University of Medicine and Pharmacy “Victor Babes”, 300041 Timisoara, Romania; florin.topala@gmail.com (F.T.); faur_andy@yahoo.com (A.F.); ajivanescu@yahoo.com (A.J.)
*
Correspondence: lucianag@umft.ro; Tel.: +40744273092
How to cite: Goguţā, L.M.; Topalā, F.; Faur, A.; Jivānescu, A. Influence of the Printing Angle on the Accuracy of 3D-Printed Interim Fixed Partial Dentures: An In Vitro Study. Timisoara Med. 2023, 2023(1), 5; doi:10.35995/20230105.
Received: 21 May 2023 / Accepted: 28 June 2023 / Published: 4 July 2023
Abstract
:Background: The aim of this study was to assess the accuracy of printed interim fixed partial dentures (i-DPSs) fabricated on 3D-printed models using different printing angles. Materials and Methods: Six printing angles were chosen, namely 90°, 75°, 60°, 45°, 30° and 15°, to fabricate sixty i-DPSs, ten for each. The measurements were performed at 100 points cervical and 100 points axial occlusal. Statistical analyses were carried out using Med Calc software and the Kolmogorov–Smirnov and Kruskal–Wallis tests. Results: The marginal fit ranged between 39.302 µm and 74.470 µm, with the best values for the 60° (39.302 µm) and 30° (43.287 µm) printing angles. The mean values for the internal fit were between 72.876 µm and 114.26 µm, with the best values for the 30° (72.876 µm) and 60° (78.049 µm) printing angles. The Kruskal–Wallis test returned a p value of p < 0.0001.
Keywords:
fixed partial dentures; printing angle; printing accuracyIntroduction
At present, research is showing an increase in the 3D printing digital workflow in fixed partial denture (FPD) manufacturing in traditional and implant prosthodontics, using different materials like ceramic nanocomposite materials, resins and laser sintering or melting metals [1,2,3,4,5,6,7,8].
For years, milling machines have been the gold standard for CAD/CAM in dentistry, especially for the restorative field because of their impressive accuracy. However, their hefty price tag makes them cost-prohibitive for many dental labs and offices. Available at a fraction of the cost without sacrificing quality, accuracy or material versatility, 3D printing can be used as a tool for restorative dentistry. Furthermore, 3D-printed FPDs have been proven to be more advantageous economically compared to subtractive manufacturing methods and, most of the time, similar in precision [9,10,11,12].
Additive manufacturing (AM) is comparable with subtractive manufacturing (SM) in terms of mechanical properties, especially with polymeric materials. However, the flexural strength of AM-printed prostheses is lower than that using conventional and SM techniques, as are the parameters of hardness and fracture load, while the marginal accuracy is similar to that of SM and conventional techniques [13,14,15,16].
The accuracy of FPDs fabricated in a digital workflow is influenced by many parameters: (1) the scanning, which can be performed directly in the patient’s oral cavity, or the prosthodontist can take an impression, pour a stone cast and scan the cast extra-orally [17]; (2) the software used for the digital design and also the skills of the dentist or dental technician in using the digital tool; (3) the materials (resistance, color stability) [18,19] and the 3D printer used for the interim FPD fabrication as well as the printing angles, which can have a significant effect on the fracture strength of three-unit interim fixed dental prostheses [16,20].
The aim of this study was to assess the accuracy of 3D-printed interim FPDs (i-DPDs) fabricated on 3D-printed models using different printing angles. The null hypothesis was that the angle of printing does not influence the accuracy (marginal and internal fits) of i-FPDs.
Materials and Methods
On a typodont (Frasaco, ANA4- Germany), the mandibular left first premolar and the left first molar were prepared with a chamfer finishing line, with an occlusal reduction of 2 mm and a 6 ° taper, by using a preparation and finishing kit (Komet-Brasseler, Lemgo, Germany). The second premolar was removed, and the gap was closed with putty polyvinylsiloxane (Variotime, Easy Putty, Kulzer, Germany). This model was scanned with an intraoral scanner (Medit I 700, MEDIT corp. Seongbuk-gu, Seoul Korea), and sixty models were printed (3D Prusa SL1S 3D printer Prusa Research a.s. Czech Republic) (Figure 1).
One printed model was scanned with the same scanner, and a three-unit FPD was designed on this model with CAD software (Exocad, Headquarters Germany exocad GmbH). The virtual space for the cement layer was set at 50 µm.
For the 3D printing of the i-FPDs, six printing angles were chosen: 90°,75°, 60°, 45°, 30° and 15°. For each angle, 10 fixed i-FPDs were fabricated (Figure 2).
Each fixed partial denture was cemented using glass ionomer cement (Ketac Molar Easy Mix, 3M Espe, Germany) and pressed for 15 minutes with the same force (300 N) using a hydraulic dental press (A5701 Italy) (Figure 3).
The samples were embedded in auto-polymerized acrylic resin (Duracryl Plus, Spofa, Czech Republic) in custom-made resin boxes (Figure 4).
After the curing of the auto-polymerized acrylic resin, the samples were cut into 3 equal slices (in a buccal–oral direction) with diamond discs and finished (Komet-Brasseler, Lemgo, Germany).
The samples were analyzed using an optical microscope (×100), and the measurements were performed with ImageJ software. The measurements were carried out on each external face, for each sample, at 100 points cervical (marginal fit/marginal gap) and 100 points axial–occlusal (internal fit/internal gap) (Figure 5).
Statistical Analysis
Statistical software (MedCalc) was used for the statistical data analysis. The data were imported into the software, and the numerical data were explored for normality by checking the data distribution using the Kolmogorov–Smirnov test.
A Kruskal–Wallis nonparametric statistical test was also used to further asses the data.
Results
The results indicated that, regarding the marginal fit, the 60° angle group displayed the best average values of 39.302 µm, being closely followed by the 30° angle group with an average value of 43.287 µm. The 45° angle group displayed an average value of 73.404 µm, followed by the 75° angle group with an average value of 66.137 µm, and the 90° angle group with an average value of 66.169 µm, while the 15° angle group displayed the least satisfactory values with an average value of 74.470 µm (Figure 6).
Regarding the internal fit, the 30° angle group displayed an average value of 72.876 µm, the 15° angle group displayed an average value of 78.801 µm, the 60° angle group displayed an average value of 78.049 µm, the 90° angle group displayed an average value of 79.122 µm, the 75° angle group displayed an average value of 83.431 µm, and the 45° angle group displayed an average value of 114.26 µm. The overall occlusal adaptation showed close values for all the groups and generally satisfactory values, especially for the 30° angle group (Figure 7).
The statistical analysis using Med-Calc and the Kolmogorov–Smirnov test showed that the data were nonparametric. The p value of the statistical significance level was set to p = 0.05.
The Kruskal–Wallis test returned a p value of p < 0.0001, rejecting the null hypothesis and showing that the results are of strong statistical relevance.
Discussion
The understanding of the factors involved in the additive manufacturing workflow leads to printing success and better outcomes for additively manufactured dental devices [21]. The printing parameters, printing structures, slicing methods and post-processing techniques significantly influence the surface roughness, printing accuracy and mechanical properties of manufactured dental devices; however, the optimization of each one may vary depending on the clinical application of the additively manufactured device.
The printing mode, resin and additive manufacturing technology used significantly influence the manufacturing accuracy of interim FDPs, particularly in the marginal area [22,23].
Marginal and internal gaps less than 100 µm are considered to be clinically acceptable. Some recent studies showed that the marginal and internal fits of FPDs manufactured using additive and subtractive methods were clinically acceptable [7,19]. The present study came to the same conclusion, showing that most fabricated 3D-printed i-FPDs had a clinically acceptable marginal fit (Figure 6) and internal fit (Figure 7). Surprisingly, the only group with a clinically unacceptable internal gap was the 45° angle group, which displayed an average value of 114.26 µm (internal fit).
Most researchers recommend the 45° angle as the best in terms of accuracy [23,24], while others recommend the more vertical (90°) printing positions [25]. One study concluded that the fit of printed metal FDPs was affected by the build orientation but remained clinically acceptable when using 0°, 30° and 45° printing angles [26].
In the present study, the results of the marginal gap showed that the best results were obtained when using the 60° printing angle (39.302 µm), which was closely followed by the 90° (66.169 µm) printing angle; therefore, this study is completely in agreement with the 90° recommendation. In all the groups, the internal gap was clinically acceptable (<100 µm), except for the 45° angle group; thus, the present study does not agree with the 45° printing angle.
In the present study, the best result (72.876 µm internal gap) was obtained when the 30° printing angle was used.
Although this study has its limits, like the use of a single resin and a single 3D printer, the null hypothesis was rejected. The accuracy (marginal and internal fits) was influenced by the printing angle.
In vitro studies have limited clinical value; therefore, to obtain stronger scientific evidence, clinical studies regarding the accuracy and mechanical and esthetic behavior of printed i-FPDs are recommended.
Conclusions
Within the limits of the present study, the best results regarding the marginal and internal fits of a printed interim fixed partial denture were obtained when the 30° and 60° printing angles were used.
Author Contributions
Conceptualization, L.M.G. and F.T.; Methodology, F.T.; Software, F.T. and A.F.; Validation, F.T., L.M.G. and A.J.; Formal Analysis, A.J.; Writing—Original Draft Preparation, L.M.G.; Writing—Review and Editing, L.M.G.; Supervision, A.J.
Acknowledgments
This study was conducted in the Research Center TADERP Timisoara, Faculty of Dentistry, University of Medicine and Pharmacy “Victor Babes” Timisoara, Romania.
Conflicts of Interest
This study was presented previously at the 45th European Prosthodontic Association Congress, 2022, Sienna, Italy (oral presentation).
References
- Joda, T.; Ferrari, M.; Gallucci, G.O.; Wittneben, J.G.; Brägger, U. Digital technology in fixed implant prosthodontics. Periodontology 2000 2017, 2, 178–192. [Google Scholar] [CrossRef]
- Joda, T.; Zarone, F.; Ferrari, M. The complete digital workflow in fixed prosthodontics: A systematic review. BMC Oral Health 2017, 2, 124. [Google Scholar] [CrossRef] [PubMed]
- Bukhari, S.; Goodacre, B.J.; AlHelal, A.; Kattadiyil, M.T.; Richardson, P.M. Three-dimensional printing in contemporary fixed prosthodontics: A technique article. J. Prosthet. Dent. 2018, 2, 530–534. [Google Scholar] [CrossRef]
- Pillai, S.; Upadhyay, A.; Khayambashi, P.; Farooq, I.; Sabri, H.; Tarar, M.; Lee, K.T.; Harb, I.; Zhou, S.; Wang, Y.; Tran, S.D. Dental 3D-Printing: Transferring Art from the Laboratories to the Clinics. Polymers 2021, 2, 157. [Google Scholar] [CrossRef] [PubMed]
- Solís Pinargote, N.W.; Smirnov, A.; Peretyagin, N.; Seleznev, A.; Peretyagin, P. Direct Ink Writing Technology (3D Printing) of Graphene-Based Ceramic Nanocomposites: A Review. Nanomaterials 2020, 10, 1300. [Google Scholar] [CrossRef]
- Jockusch, J.; Özcan, M. Additive manufacturing of dental polymers: An overview on processes, materials and applications. Dent. Mater. J. 2020, 2, 345–354. [Google Scholar] [CrossRef] [PubMed]
- Revilla-León, M.; Sadeghpour, M.; Özcan, M. An update on applications of 3D printing technologies used for processing polymers used in implant dentistry. Odontology 2020, 108, 331–338. [Google Scholar] [CrossRef]
- Goguta, L.; Lungeanu, D.; Negru, R.; Birdeanu, M.; Jivanescu, A.; Sinescu, C. Selective Laser Sintering versus Selective Laser Melting and Computer Aided Design—Computer Aided Manufacturing in Double Crowns Retention. J. Prosthodont. Res. 2021, 2, 371–378. [Google Scholar] [CrossRef]
- Dentistry Today. Available online: https://www.dentistrytoday.com/2021-the-year-of-3d-printing-in-dentistry/Zimmermann (accessed on 22 June 2022).
- Zimmermann, M.; Ender, A.; Egli, G.; Özcan, M.; Mehl, A. Fracture load of CAD/CAM-fabricated and 3D-printed composite crowns as a function of material thickness. Clin. Oral Investig. 2019, 6, 2777–2784. [Google Scholar] [CrossRef] [PubMed]
- Haddadi, Y.; Ranjkesh, B.; Isidor, F.; Bahrami, G. Marginal and internal fit of crowns based on additive or subtractive manufacturing. Biomater. Investig. Dent. 2021, 2, 87–91. [Google Scholar] [CrossRef] [PubMed]
- Huang, G.; Wu, L.; Hu, J.; Zhou, X.; He, F.; Wan, L.; Pan, S.T. Main Applications and Recent Research Progresses of Additive Manufacturing in Dentistry. BioMed Res. Int. 2022, 2022, 5530188. [Google Scholar] [CrossRef] [PubMed]
- Valenti, C.; Isabella Federici, M.; Masciotti, F.; Marinucci, L.; Xhimitiku, I.; Cianetti, S.; Pagano, S. Mechanical properties of 3D-printed prosthetic materials compared with milled and conventional processing: A systematic review and meta-analysis of in vitro studies. J. Prosthet. Dent. 2022, 5, S0022-3913(22)00415-2. [Google Scholar] [CrossRef]
- Othman, A.; Ströbele, D.; Lüllmann, A.; Stehle, O.; Alevizakos, V.; von See, C. Comparative mechanical testing for the digitally produced provisional fixed partial denture prostheses to the conventional method: In-vitro study. Int. J. Prosthodont. 2021. [Google Scholar] [CrossRef]
- Henderson, J.Y.; Korioth, T.V.; Tantbirojn, D.; Versluis, A. Failure load of milled, 3D-printed, and conventional chairside-dispensed interim 3-unit fixed dental prostheses. J. Prosthet. Dent. 2022, 127, 275.e1–275.e7. [Google Scholar] [CrossRef] [PubMed]
- Turksayar, A.A.; Donmez, M.B.; Olcay, E.O.; Demirel, M.; Demir, E. Effect of printing orientation on the fracture strength of additively manufactured 3-unit interim fixed dental prostheses after aging. J. Dent. 2022, 2022, 104155. [Google Scholar] [CrossRef] [PubMed]
- Amornvit, P.; Rokaya, D.; Sanohkan, S. Comparison of Accuracy of Current Ten Intraoral Scanners. BioMed Res. Int. 2021, 2021, 2673040. [Google Scholar] [CrossRef] [PubMed]
- Karasan, D.; Legaz, J.; Boitelle, P.; Mojon, P.; Fehmer, V.; Sailer, I. Accuracy of Additively Manufactured and Milled Interim 3-Unit Fixed Dental Prostheses. J. Prosthodont. 2022, 2, 58–69. [Google Scholar] [CrossRef] [PubMed]
- Song, G.; Son, J.W.; Jang, J.H.; Choi, S.H.; Jang, W.H.; Lee, B.N.; Park, C. Comparing volumetric and biological aspects of 3D-printed interim restorations under various post-curing modes. J. Adv. Prosthodont. 2021, 2, 71–78. [Google Scholar] [CrossRef] [PubMed]
- Alharbi, N.; Osman, R.; Wismeijer, D. Effects of build direction on the mechanical properties of 3D-printed complete coverage interim dental restorations. J. Prosthet. Dent. 2016, 2, 760–7. [Google Scholar] [CrossRef]
- Piedra-Cascón, W.; Krishnamurthy, V.R.; Att, W.; Revilla-León, M. 3D printing parameters, supporting structures, slicing, and post-processing procedures of vat-polymerization additive manufacturing technologies: A narrative review. J. Dent. 2021, 2, 103630. [Google Scholar] [CrossRef]
- Yao, Q.; Morton, D.; Eckert, G.J.; Lin, W.S. The effect of surface treatments on the color stability of CAD-CAM interim fixed dental prostheses. J. Prosthet. Dent. 2021, 2, 248–253. [Google Scholar] [CrossRef] [PubMed]
- Almejrad, L.; Yang, C.C.; Morton, D.; Lin, W.S. The Effects of Beverages and Surface Treatments on the Color Stability of 3D-Printed Interim Restorations. J. Prosthodont. 2022, 2, 165–170. [Google Scholar] [CrossRef] [PubMed]
- Thakur, J.; Parlani, S.; Shivakumar, S.; Jajoo, K. Accuracy of marginal fit of an implant-supported framework fabricated by 3D printing versus subtractive manufacturing technique: A systematic review and meta-analysis. J. Prosthet. Dent. 2021, 129, 301–309. [Google Scholar] [CrossRef] [PubMed]
- Rubayo, D.D.; Phasuk, K.; Vickery, J.M.; Morton, D.; Lin, W.S. Influences of build angle on the accuracy, printing time, and material consumption of additively manufactured surgical templates. J. Prosthet. Dent. 2021, 126, 658–663. [Google Scholar] [CrossRef] [PubMed]
- Daou, E.E. Does the Build Orientation Parameter Affect the Fit of Fixed Partial Dentures Fabricated by Additive Manufacturing? Int. J. Prosthodont. 2022. [Google Scholar] [CrossRef]
Figure 1.
Three-dimensionally printed models.
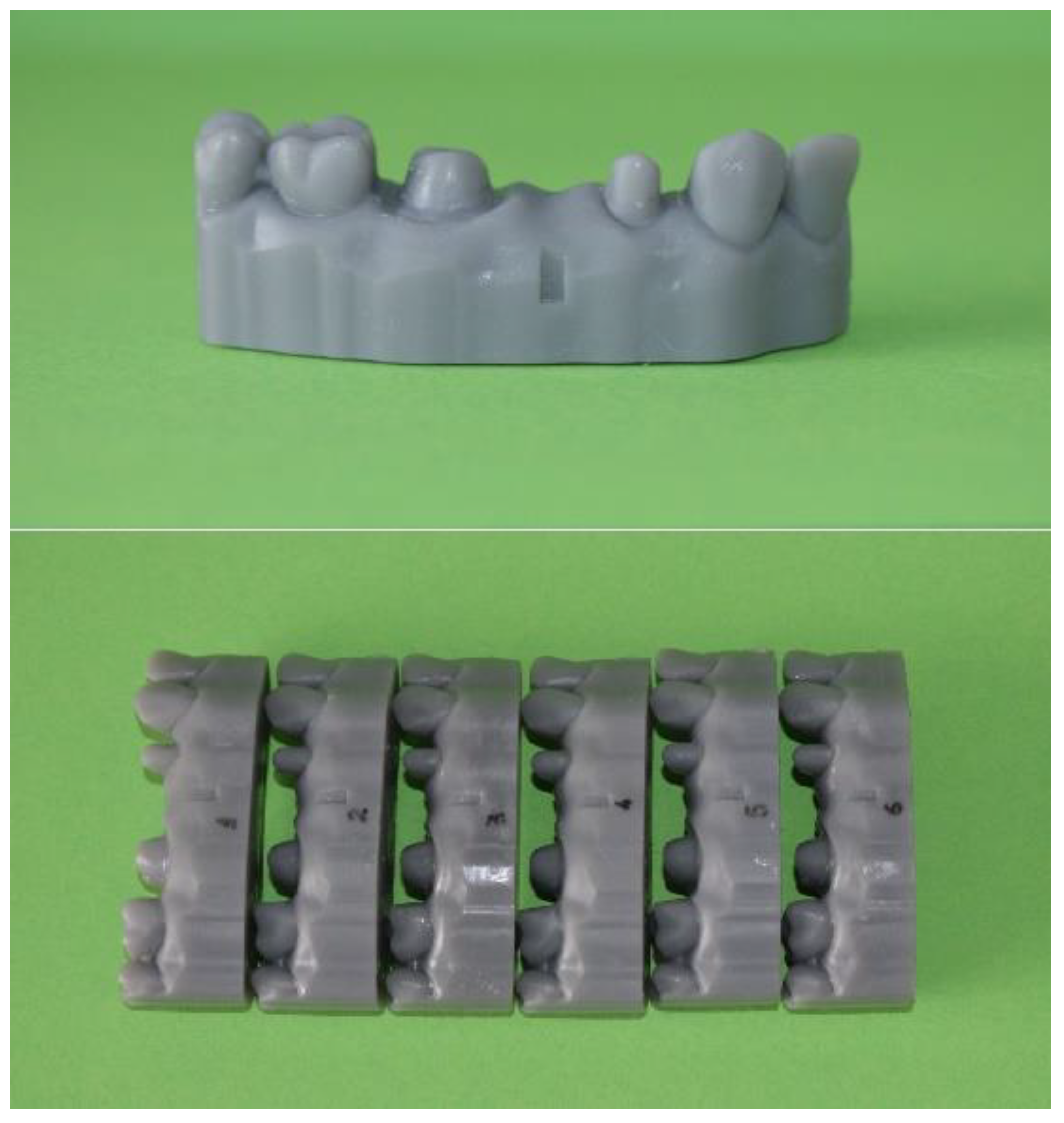
Figure 2.
Three-dimensionally printed i-FPDs using different printing angles.
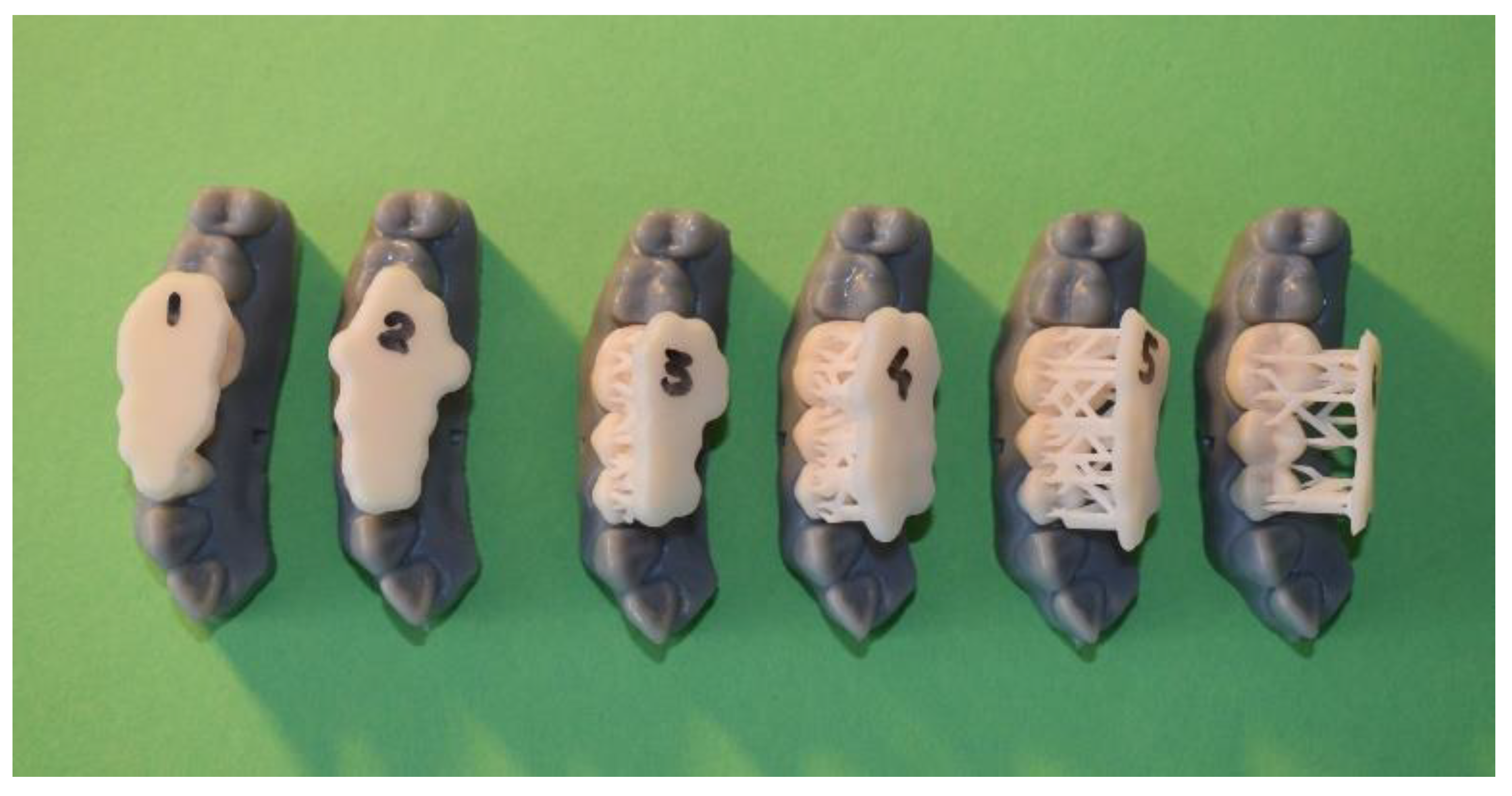
Figure 3.
Printed i-FPDs cemented on the 3D-printed models.
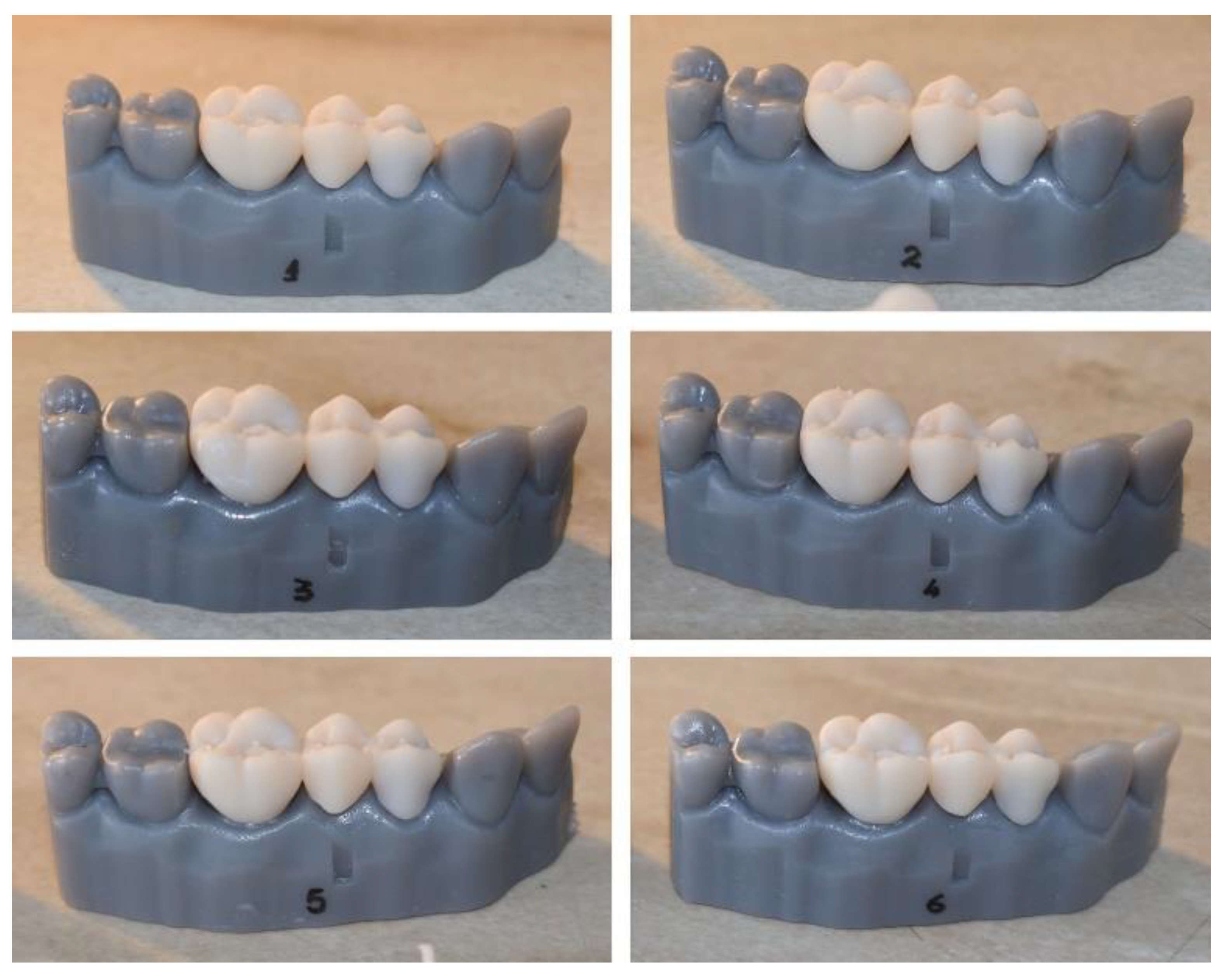
Figure 4.
The samples embedded in auto-polymerized acrylic resin.
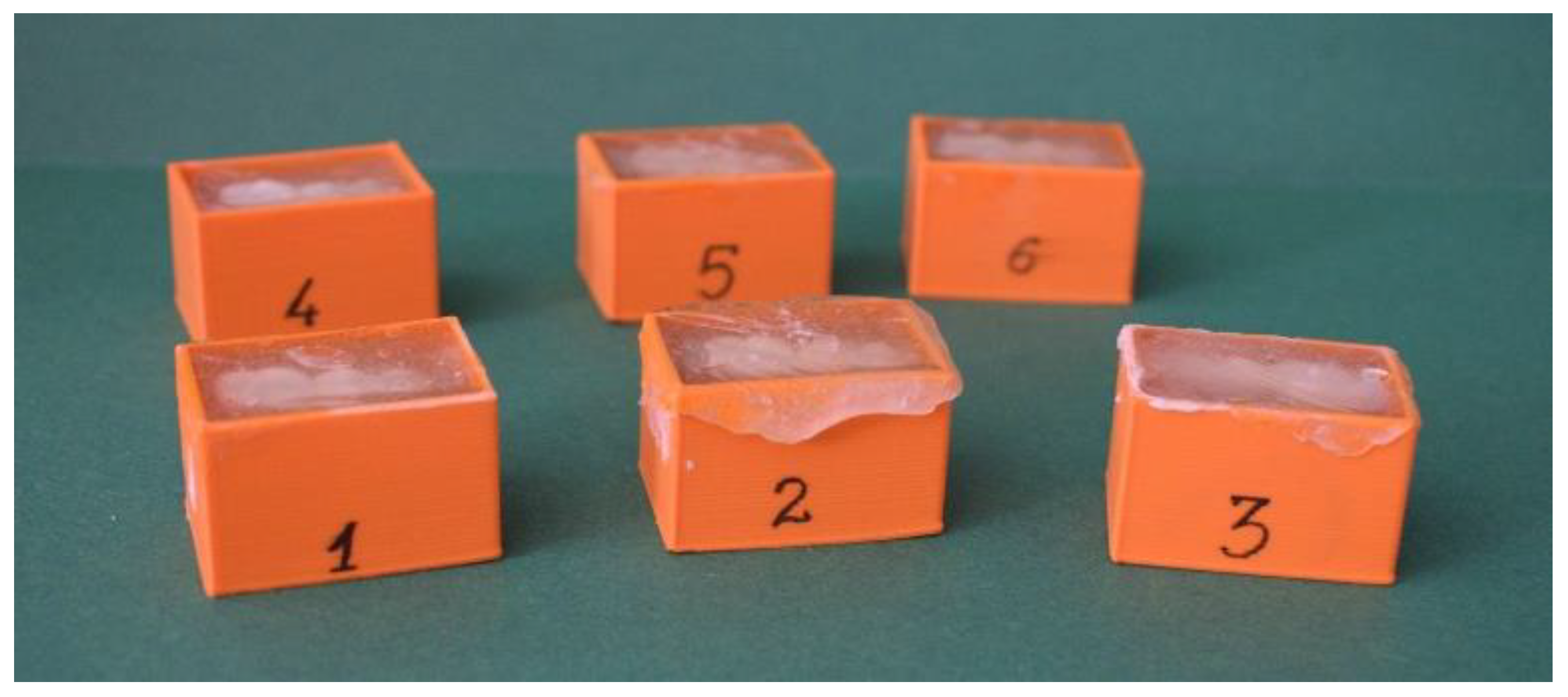
Figure 5.
Microscopic images (×100) of the marginal and internal gaps.
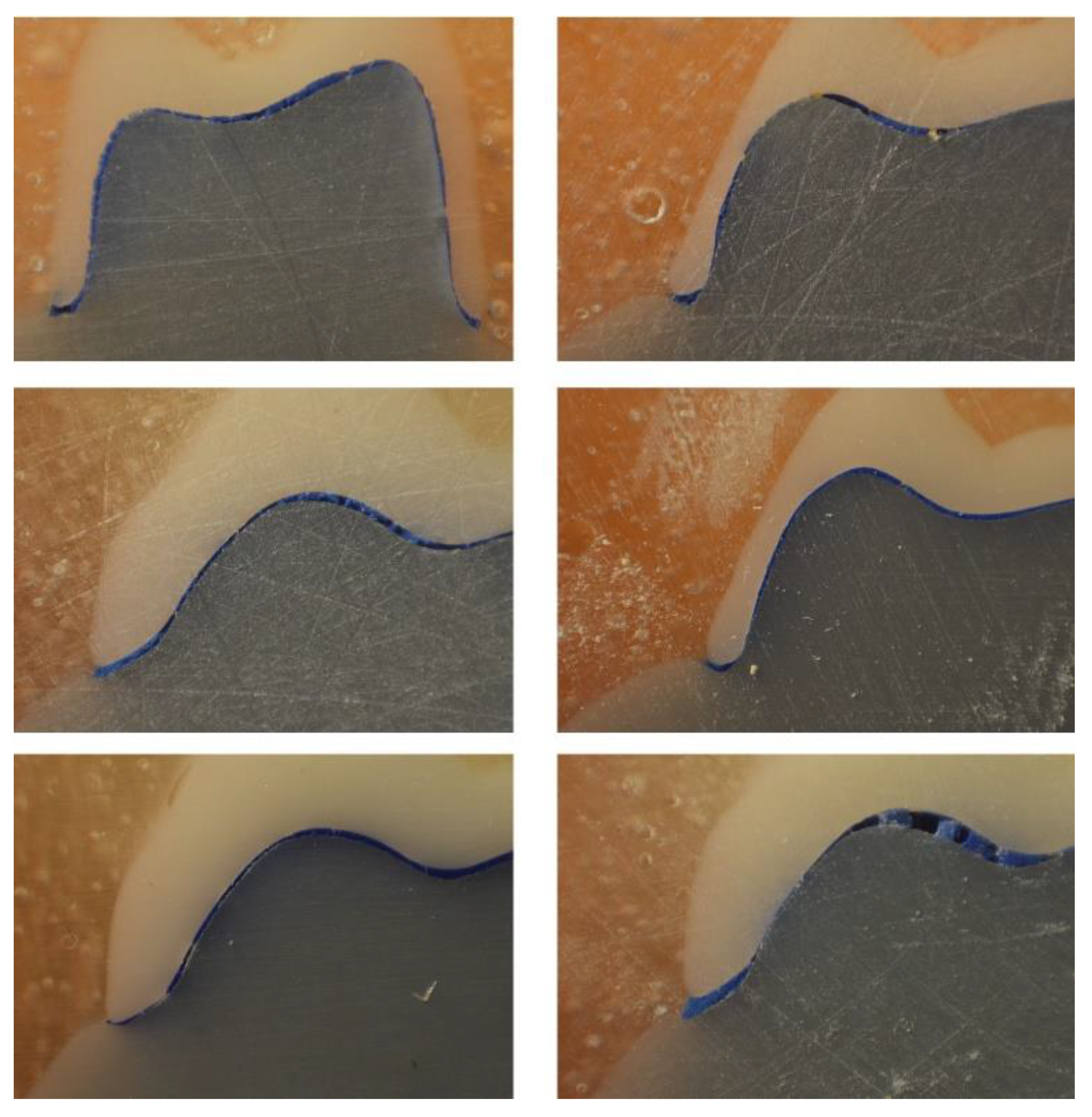
Figure 6.
Kruskal–Wallis test for the marginal gap.
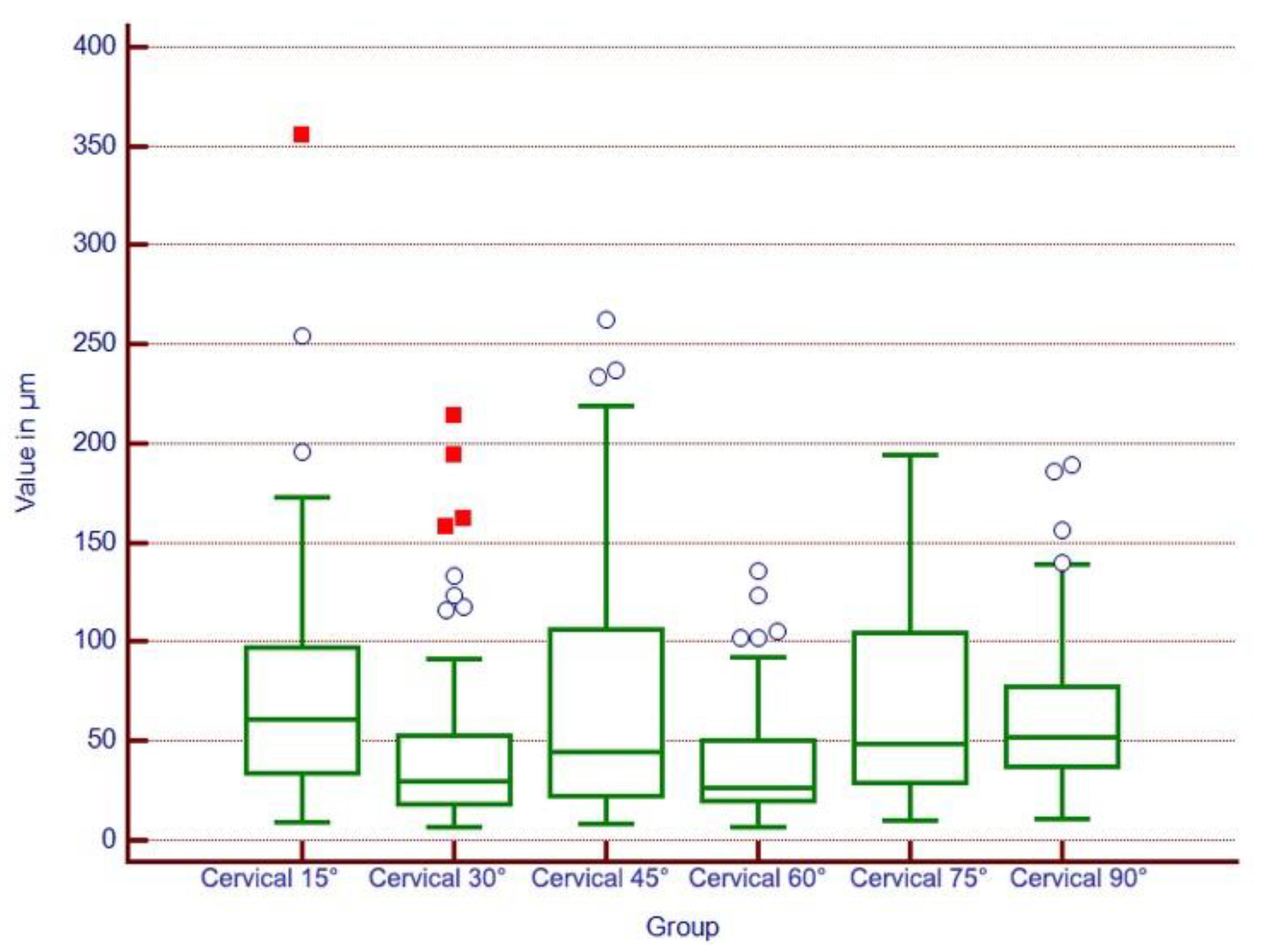
Figure 7.
Kruskal–Wallis test for the internal gap.
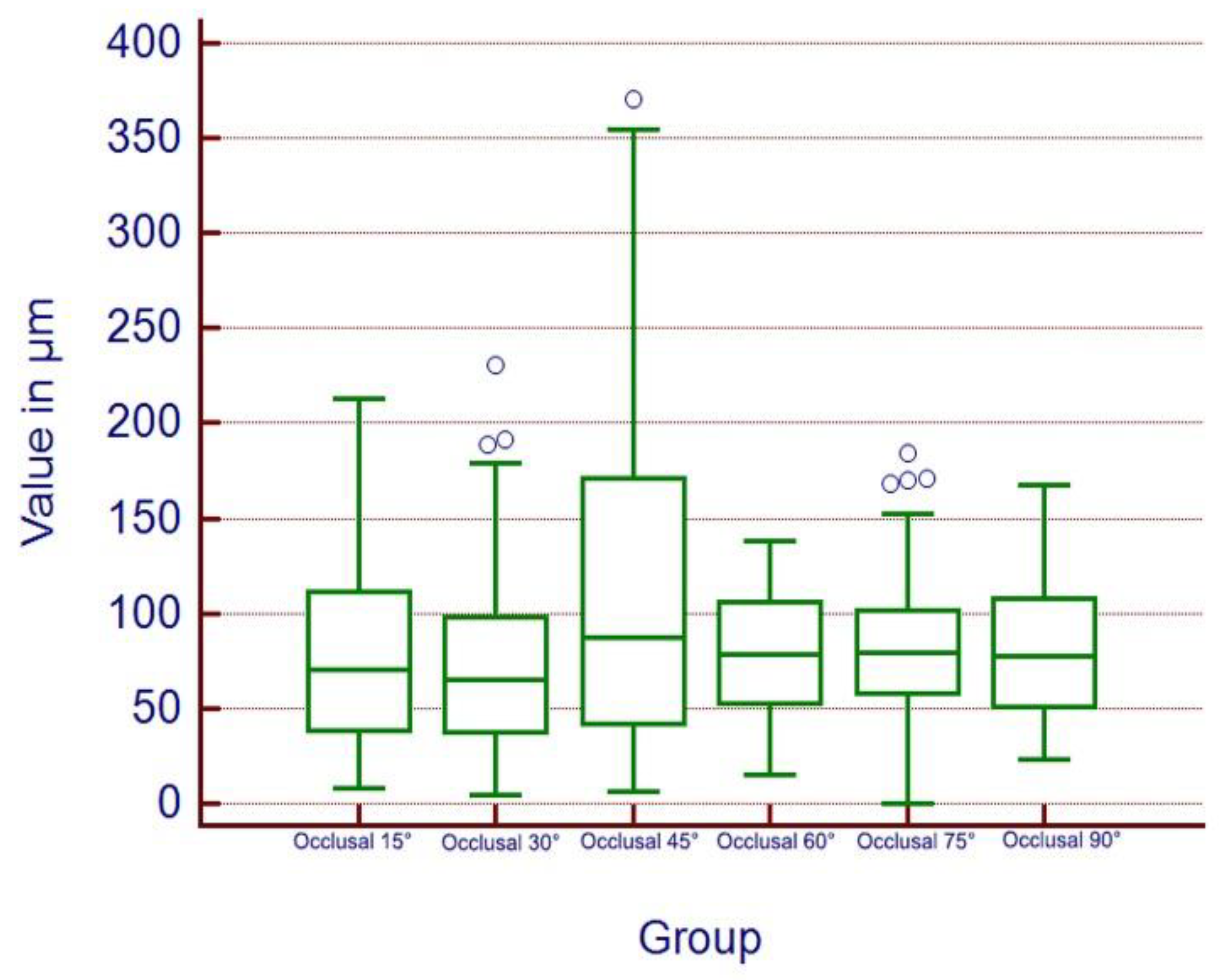
© 2023 Copyright by the authors. Licensed as an open access article using a CC BY 4.0 license.